Dongxiao Zhang1,2, Meghan F. Cronin2, Xiaopei Lin3, Ryuichiro Inoue4, Andrea J. Fassbender5, Stuart P. Bishop6, Adrienne Sutton2
1. University of Washington
2. NOAA Pacific Marine Environmental Laboratory
3. Ocean University of China, China
4. Japan Agency for Marine-Earth Science and Technology, Japan
5. Monterey Bay Aquarium Research Institute
6. North Carolina State University
Western boundary currents (WBCs) and their extensions (WBCE) are characterized by intense air-sea heat, momentum, buoyancy, and carbon dioxide (CO2) fluxes (Figure 1a). These large ocean-atmosphere exchanges contribute to the global balance of physical and biogeochemical ocean properties. Excess heat absorbed by the ocean in the tropics is transported poleward, mainly by WBCs (Trenberth and Solomon 1994; Fillenbaum et al. 1997; Zhang et al. 2001; Johns et al. 2011), and then released back to the atmosphere along the WBCs and their extensions in subtropical mid-latitudes at the subtropical and subarctic ocean boundaries (Figure 1a). For this reason, WBC regions are referred to as climatic “hot spots” (Nakamura et al. 2015). Likewise, WBC regions are ocean carbon hot spots, areas of large CO2 uptake that counterbalance the large CO2 outgassing in the tropics (Figure 1b). For OceanObs’09, Cronin et al. (2010) made strong recommendations to include multidisciplinary observations in WBC regional observing systems. The present need for such a system is more urgent than ever. While many open questions remain regarding the role of eddies in ventilation and mode water formation events and their interaction with the biological pump, new technologies are making multidisciplinary observations at these scales more feasible than ever. Use of these new tools during process studies could help to address many of the remaining open questions in WBC regions. OceanObs’19 is two years away, making it timely to review the present observing system for WBC regions and begin strategizing the necessary improvements for the next decade.
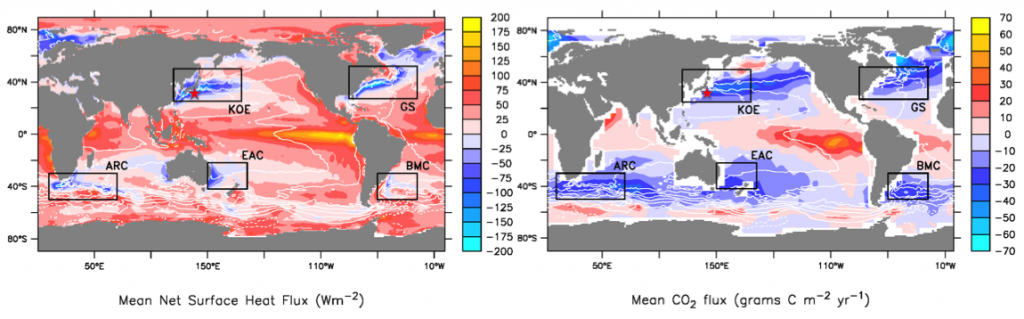
Figure 1. Annual mean air-sea net surface heat flux into the ocean from objectively analyzed flux (OAFlux; Yu and Weller 2007) and sea-to-air surface CO2 flux from Takahashi et al. (2009). White contours are the mean dynamic sea level (Rio and Hernandez 2004); star is the NOAA/PMEL KEO location. The WBC regions in the boxes are the Kuroshio-Oyashio Extension (KOE), Gulf Stream (GS), Brazil and Malvinas Currents (BMC), East Australian Current (EAC), and Agulhas Return Current (ARC). Figure adapted from Cronin et al. (2010).
The following sections briefly describe requirements of the ocean observing system in WBC regions, and how the current or planned observing system is meeting these requirements, such as international partnerships and collaborations. We also briefly discuss some underway process studies and raise some questions that still need to be addressed, by using the Kuroshio Extension observing system as an example that is applicable to other WBC regions. The goal of this article is to motivate discussion for developing future WBC regional observing systems in preparation for OceanObs’19.
Multiscale multidisciplinary air-sea interaction in WBC regions
Following the principles of the Framework for Ocean Observing (Lindstrom et al. 2012), the first step in developing an “observing system that is fit for purpose” is to define the observational requirements of the system, keeping in mind that these can only be fulfilled by an integrated system that spans multiple time and space scales.
A key characteristic of WBC regions is that their intense air-sea fluxes are associated with strong fronts and energized mesoscale and submesoscale eddies. Strong air-sea heat fluxes effectively project the SST front into the atmosphere, potentially affecting storm tracks and mid-latitude weather (Minobe et al. 2008; Small et al. 2008; Kwon et al. 2010). Carbon uptake in the WBCs is largely controlled by physical processes associated with wintertime heat loss that decreases both SST and surface water pCO2 and increases the ocean’s thermodynamic drive to absorb atmospheric CO2. Furthermore, winter heat loss in WBC regions leads to subduction and the formation of mode waters (Qiu et al. 2006; Cronin et al. 2013; Oka et al. 2015) that transport the absorbed CO2 to the ocean interior and act as an important anthropogenic CO2 sink pathway (Sabine et al. 2004). However, the biological pump also plays an important role in determining the magnitude of natural carbon uptake in the transition region between subtropical and subarctic waters (Takahashi et al. 2009; Fassbender et al. 2017; Wakita et al., 2016). Primary production and ecosystem structure and function are strongly regulated by the energetic fronts and (sub)mesoscale eddies associated with WBCs that supply nutrients to the euphotic zone via upwelling (McGillicuddy 2016; Mahadevan 2016; and the references therein) and cross-frontal exchange between high-nutrient, cold subarctic waters and low-nutrient, warm subtropical waters in the upper ocean (Ayers and Lozier 2012; Nagano et al. 2016; Nagai and Clayton 2017). Questions remain about the importance of these biological processes in local anthropogenic carbon uptake relative to other large-scale chemical and physical processes, such as changes in the seawater buffer capacity and mode and intermediate water formation rates. Within the ocean and atmosphere physics communities, it is becoming clear that to properly represent the energy balance of the climate system in a WBC region, it is necessary to resolve the multi-scale ocean and atmosphere interactions, from large-scale to frontal scale to mesoscale, and potentially even submesoscale. As discussed at the recent joint US CLIVAR and Ocean Carbon and Biogeochemistry (OCB) Ocean Carbon Hot Spots workshop, this is less clear for the biogeochemical system. While biological production is clearly sensitive to fronts and eddies – and recent data from the Kuroshio Extension Observatory (KEO) sediment trap show an accumulation peak that can be traced back to a cold-core eddy (M. Honda, pers. comm. 2017) – physical controls of solubility and buffer capacity may be more important for the carbon cycle. Participants of the Ocean Carbon Hot Spots workshop discussed the challenge of quantifying CO2 uptake and understanding air-sea exchange processes in WBC regions in the face of an incomplete observing system and lack of coverage across the fronts and eddies in these systems. Process studies will enable us to examine the complex relationships between air-sea fluxes, eddy activity, mode water formation, physical and biogeochemical properties of mode waters, and primary production. An improved understanding of these physical-chemical-biological interactions can reveal important information about the underlying mechanisms driving low-frequency decadal variations and gyre-scale circulation in WBC regions. These multi-scale, coupled interactions must be considered in the development of a more comprehensive “fit for purpose” WBC regional observing system.
Observing systems of WBC regions
Satellites represent a critical component of all observing systems, delivering global coverage. Because WBC regional fronts are often associated with clouds and rain that can disrupt satellite remote sensing, some remotely sensed fields could have systematic biases in frontal regions. Thus, the WBC regional observing system plan must include efforts to avoid biases and aliasing from improperly resolved fronts and eddies.
Strong currents, winter storms, and warm season typhoons and hurricanes can also make WBC regions challenging for in situ observations. Of all the WBC regions, the Kuroshio Extension currently has the most complete observing system, so we focus on this system as a potential roadmap for other systems.
Kuroshio Extension Observatory: NOAA surface mooring and JAMSTEC sediment trap
One of the most important observing system components in the Kuroshio Extension is NOAA’s long-term climate reference station, KEO. KEO is strategically located in the Kuroshio Extension recirculation gyre at 32.3°N, 144.5°E (star in Figure 1) on the warm side of the Kuroshio front, an ideal region for monitoring the air-sea interactions that result in mode water formation through winter and spring. Additionally, the site is frequently visited by typhoons during summer and early fall, providing case studies of air-sea interactions between warm water and strong storms. Since 2004, NOAA/PMEL’s Ocean Climate Stations group has maintained a surface mooring at KEO. The NOAA surface mooring measures the meteorological, biogeochemical, and physical ocean variables for estimating air-sea exchanges of heat, moisture, momentum, and carbon dioxide; ocean acidification; and upper ocean variability associated with air-sea interaction. Data are freely available in real time (Figure 2) and are available on GTS (Global Telecommunication System) for improving numerical weather prediction and ocean-atmosphere reanalysis.
Since 2014, Honda et al. (JAMSTEC) have maintained a sediment trap mooring at KEO (Honda pers. comm. 2017). Prior to this, the sediment trap had been deployed at the Japanese S1 mooring, located southeast of KEO at 30°N, 145°E (Honda et al. 2017). The deep sediment trap at 5000 m (800 m above sea floor) positioned next to the KEO surface mooring provides crucial information about the processes affecting nutrient supply that supports ocean productivity and biological carbon export in this subtropical oligotrophic region.
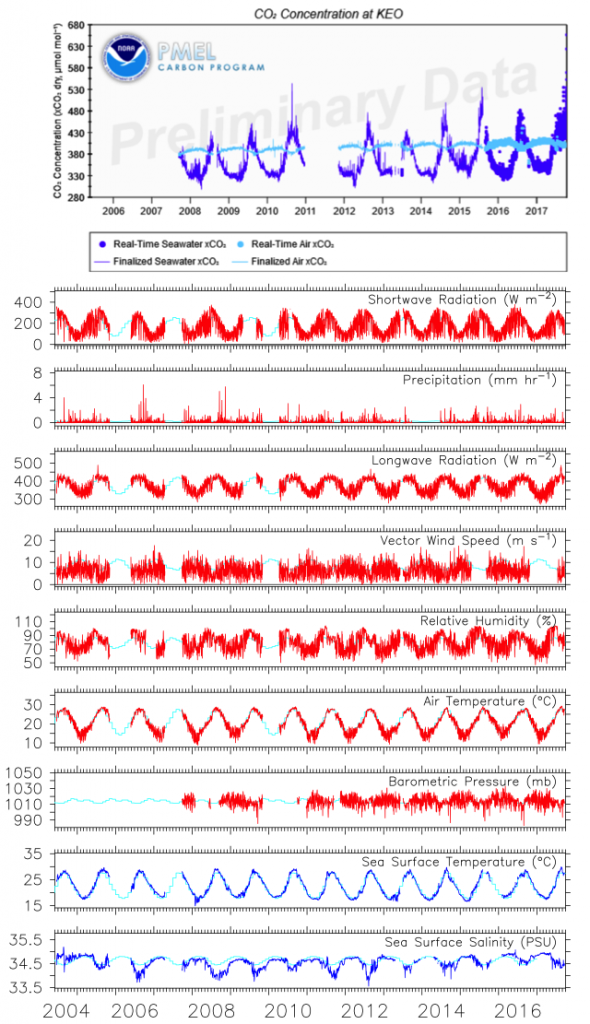
Figure 2. An example of KEO surface observations from real-time data display and delivery web pages.
Japanese-funded process studies JKEO, Hot-Spot, and INBOX
Over the past 13 years, there have been a number of process studies in the Kuroshio Extension region. Most notably, the US-funded Kuroshio Extension System Study (KESS) focused on the dynamics of the mesoscale meanders on the Kuroshio Extension and their interaction with the recirculation gyres north and south of the jet (Jayne et al. 2009; Donohue et al. 2008). This was followed by a study of the effect of the Kuroshio Extension front on the air-sea fluxes and interactions, through deployment of a JAMSTEC-KEO (JKEO) surface mooring north of the Kuroshio Extension front paired with the NOAA KEO mooring south of the front (Konda et al. 2010). Then from 2010 to 2015, the extremely successful Japanese process study, “Multi-Scale Air-Sea Interaction under the East-Asian Monsoon: A ‘Hot Spot’ in the Climate System” (Hot-Spot), led to a large field experiment in the region that included a surface flux mooring K-TRITON buoy deployed closer to the center of the Kuroshio Extension jet, which captured the unusual mesoscale exchanges of water mass properties across the Kuroshio Extension front (Nagano et al. 2016). Another Hot-Spot study with three research vessels, each occupying a half-degree latitude between 35°N-37°N along 143°E and transiting back and forth across the Kuroshio Extension SST front (Kawai et al. 2015), showed unprecedented details of dramatic surface latent and sensible heat flux changes and the response of the deep atmospheric boundary layer across the SST front.
While the Japanese Hot-Spot experiment focused on the physical air-sea interactions, the Japanese Western North Pacific Integrated Physical-Biogeochemical Ocean Observation Experiment (INBOX) (Inoue et al. 2016a) focused on biophysical interactions. In 2011, centered around the S1 mooring in a 150-km box, 18 Argo floats equipped with dissolved oxygen sensors were deployed. In addition, a four-month Seaglider survey was conducted between S1 and KEO in 2014. Results showed a strong association between dissolved oxygen patchiness and mesoscale and submesoscale eddies. With proper coordination through CLIVAR, leveraging international research funding could expand these Japanese-funded experiments.
Chinese KEO buoy
Motivated by recent exciting findings from ultra-high-resolution coupled model simulations showing the importance of latent and sensible heat release from warm ocean eddies in forcing the atmosphere (Ma et al. 2015) and regulating the Kuroshio Extension jet (Ma et al. 2016), the Ocean University of China has successfully deployed an air-sea flux buoy, the Chinese KEO (C-KEO), north of Kuroshio Extension axis at 39ºN, 149.25ºE in October 2017. Similar to KEO, both subsurface and surface measurements at C-KEO will be transmitted and made available to the scientific community in real time when it reaches stable state. In addition, the Ocean University of China has deployed three subsurface moorings (M1: 32.4ºN, 146.2ºE; M2: 39ºN, 150ºE; and M3: 35ºN, 147.6ºE) equipped with ADCP, CTD, a current meter, and McLane Moored Profiler (M1) to monitor the subsurface eddy structure and variability. Over the past three years, they have deployed 19 Argo floats in the Kuroshio Extension region, with a cluster of floats deployed in an anticyclone eddy, providing the detailed eddy contribution to the subduction and mode water formation (Xu et al. 2016). The Qingdao National Laboratory for Marine Science and Technology, as part of its ‘Transparent Ocean’ project, support all of these observing activities.
Challenges and emerging technologies
Characterized by strong winds, high seas, and fast and deep currents, WBC regions are some of the most challenging environments to observe with moored surface buoys. KEO’s long history of success demonstrates that with careful planning, dedication, and international collaborations, sustained moored buoys can be maintained in WBC regions to provide long time series of high-frequency and high-quality simultaneous measurements of subsurface and surface variables. However, due to increased risk of breaking mooring lines in strong, deep jet streams, it is recommended that these long-term reference sites be placed outside of the strongest jet and in the recirculation gyre or northern flank of the jet, like KEO, or the former J-KEO and new C-KEO moorings.
Lagrangian floats have proven to be ideal for studying small-scale fronts and eddies (Shcherbina et al. 2014; Thomas et al. 2017; Inoue et al. 2016b; Xu et al. 2016). Newly available Biogeochemical (BGC)-Argo floats (Johnson and Claustre 2016) may be especially useful for monitoring ocean carbon hot spots to gain a more complete understanding of physical and biogeochemical processes in WBC regions. However, for sustained monitoring and quantifying heat or carbon uptake in eddy-rich WBC regions, a Lagrangian float array would have difficulty to maintain position in strong jets and is susceptible to sampling biases due to the tendency of the floats to be more likely trapped in cyclonic eddies (Rainville et al. 2014; Legg and McWilliams 2002). Controlled surveys by self-propelled autonomous underwater gliders will therefore be necessary to measure moving fronts and eddies and augment moored and Lagrangian components of the observing system.
In situ air-sea flux measurements across fronts and eddies have traditionally required research vessels or voluntary observing ships restricted to limited transit tracks (Fairall et al. 2003; Smith et al. 2016; Palevsky et al. 2016). However, new unmanned surface vehicles (USV) such as Wavegliders (Thompson and Girton 2017) and Saildrones (Meinig et al. 2015; Mordy et al. 2017) are now also being used for air-sea flux measurements of this nature. The Saildrone is especially well suited for collecting observations in the challenging sea conditions of WBC regions. Powered by wind and solar energy with average speed of 3-5 knots (depending on wind, with maximum speed of 7-8 knot), the Saildrone is two times faster than other USVs and has completed a voyage at sea lasting 12 months and covering 16,000 nautical miles. To make the Saildrone capable of observing air-sea exchange processes, NOAA/PMEL, the University of Washington, and Saildrone, Inc. have collaborated to successfully install sensors with equivalent or better quality than those currently used on tropical atmosphere and ocean (TAO) buoys for air-sea flux measurements, as well as a 300 kHz acoustic doppler current profiler (ADCP) for upper ocean current measurements. The standard Saildrone sensor suite also includes: the new PMEL autonomous surface vehicle CO2 system for air-sea CO2 flux measurements; sea surface dissolved oxygen, pH, and chlorophyll sensors; and subsurface backscatter sensing capability from the ADCP, making it a truly interdisciplinary observing platform (Figure 3). Most importantly, Saildrone deployments, measurements, and platform recoveries require no ship time. For example, two Saildrones were recently launched from San Francisco, CA with missions to the eastern tropical Pacific as part of the Tropical Pacific Observing System 2020 project (TPOS2020) and to participate in the field campaign of the NASA Salinity Processes in the Upper Ocean Regional Study (SPURS-2).
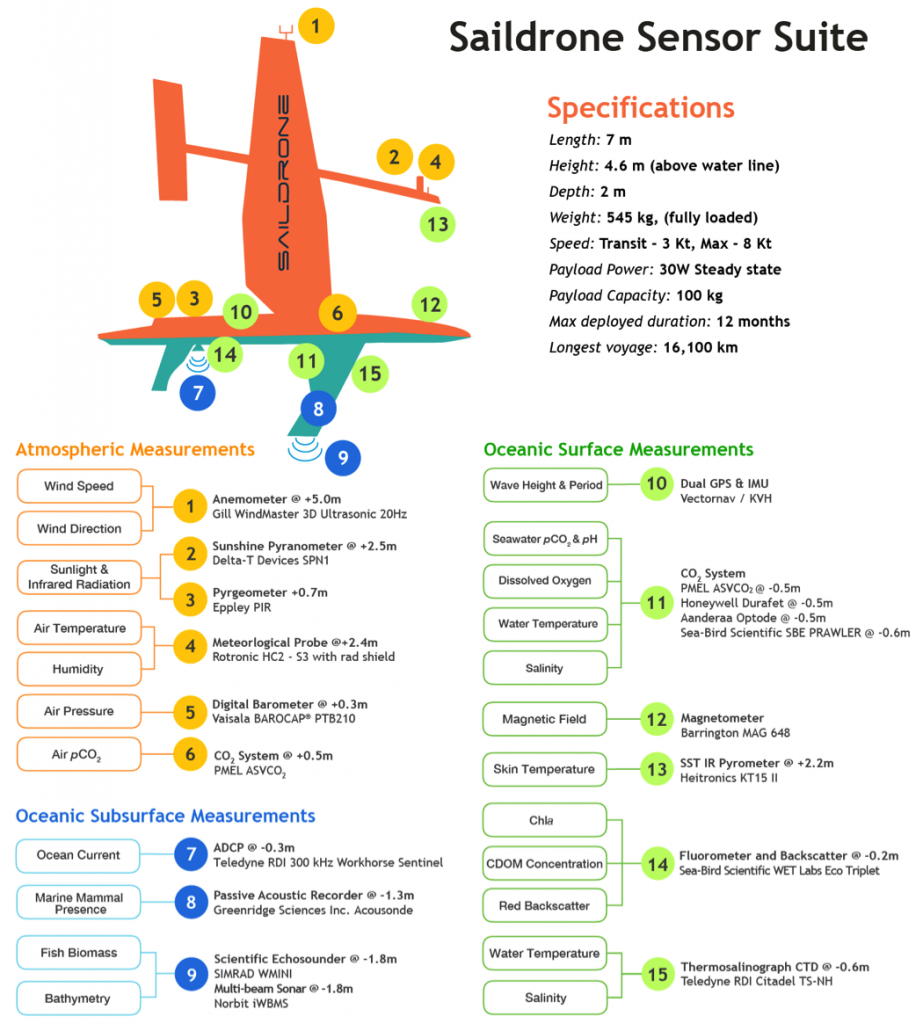
Figure 3. Physical and biogeochemical variables measured by Saildrone.
Conceptual WBC regional observing system for Ocean Obs’19
With uninterrupted satellite measurements of winds, sea surface height, SST, sea surface salinity, precipitation, and ocean color providing a large-scale context of in situ observations, a sustained WBC regional observing system should have the following components to observe multi-scale multidisciplinary processes:
- Long-term moored climate reference buoys in the upper ocean equipped with air-sea flux, physical, and biogeochemical sensors and sediment traps, preferably at the opposite flanks of the WBC extension jets
- An array of Lagrangian floats, especially BGC-Argo floats, equipped with standard biogeochemical sensors
- Underwater gliders for controlled observations of the subsurface ocean and across fronts and eddies
- Unmanned surface vehicle sections crossing WBC regional fronts and eddies around and between reference buoys
- Underway shipboard measurements including launch of weather balloons when crossing fronts and eddies during float deployments and glider operations
Before such an observing system can be fully developed, process studies are recommended to better understand key physical and biogeochemical processes operating in WBC regions and their associated temporal and spatial scales of variability. Process studies will also inform and optimize the use of newer technologies such as self-navigating platforms together with Lagrangian and Eulerian observations in WBC regions.
Acknowledgements
This is a NOAA PMEL contribution number 4725 and is partially funded by the Joint Institute for the Study of the Atmosphere and Ocean (JISAO) under NOAA Cooperative Agreement and NA15OAR4320063, Contribution No. 2017-0118.
References
Ayers, J. M., and M. S. Lozier, 2012: Unraveling dynamical controls on the North Pacific carbon sink. J. Geophys. Res., 117, doi:10.1029/2011JC007368.
Cronin, M. F., and Coauthors, 2010: Monitoring ocean-atmosphere interactions in western boundary current extensions. In Proceedings of the “OceanObs’09: Sustained Ocean Observations and Information for Society” Conference (Vol. 2), Venice, Italy, 21-25 September 2009, J. Hall, D. E. Harrison, and D. Stammer, Eds., ESA Publication WPP-306, doi:10.5270/OceanObs09.cwp.20.
Cronin, M. F., N. A. Bond, J. T. Farrar, H. Ichikawa, S. R. Jayne, Y. Kawai, M. Konda, B. Qiu, L. Rainville, and H. Tomita, 2013: Formation and erosion of the seasonal thermocline in the Kuroshio Extension recirculation gyre. Deep-Sea Res. II, 85, 62-74, doi:10.1016/j.dsr2.2012.07.018.
Donohue, K. A., and Coauthors, 2008: Program studies the Kuroshio Extension. Eos, 89, 161-162, doi: 10.1029/2008EO170002.
Fairall, C. W., E. F. Bradley, J. E. Hare, A. A. Grachev and J. B. Edson, 2003: Bulk parameterization of air–sea fluxes: Updates and verification for the COARE algorithm. J. Climate, 16, 571-591, doi: 10.1175/1520-0442(2003)016<0571:BPOASF>2.0.CO;2.
Fassbender, A. J., C. L. Sabine, M. F. Cronin, and A. J. Sutton, 2017: Mixed-layer carbon cycling at the Kuroshio Extension Observatory. Glob. Biogeochem. Cycles, 31, doi:10.1002/2016GB005547.
Fillenbaum, E. R., T. N. Lee, W. E. Johns, and R. Zantopp, 1997: Meridional heat transport variability at 26.5°N in the North Atlantic. J. Phys. Oceanogr., 27, 153–174, doi:10.1175/1520-0485(1997)027<0153:MHTVAN>2.0.CO;2.
Honda, M. C., and Coauthors, 2017a: Comparison of carbon cycle between the western Pacific subarctic and subtropical time-series stations: highlights of the K2S1 project. J. Oceanogr., 73, 647-667, doi:10.1007/s10872-017-0423-3.
Inoue, R., and Coauthors, 2016a: Western North Pacific Integrated Physical-Biogeochemical Ocean Observation Experiment (INBOX): Part 1. Specifications and chronology of the S1-INBOX floats. J. Mar. Res., 74, 43–69, doi:10.1357/002224016819257344.
Inoue, R., V. Faure, and S. Kouketsu, 2016b: Float observations of an anticyclonic eddy off Hokkaido. J. Geophys. Res: Oceans, 121, 6103–6120, doi:10.1002/2016JC011698.
Jayne, S. R., and Coauthors, 2009: The Kuroshio Extension and its recirculation gyres. Deep-Sea Res. I, 56, 2088-2099, doi:10.1016/j.dsr.2009.08.006.
Johns, W. E., and Coauthors, 2011: Continuous, array-based estimates of Atlantic Ocean heat transport at 26.5°N. J. Climate, 24, 2429-2449, doi:10.1175/2010JCLI3997.1.
Johnson, K., and H. Claustre 2016: Bringing biogeochemistry into the Argo age. Eos, 97, doi:10.1029/2016EO062427
Kawai, Y., T. Miyama, S. Iizuka, A. Manda, M. K. Yoshioka, S. Katagiri, Y. Tachibana, and H. Nakamura, 2015: Marine atmospheric boundary layer and low-level cloud responses to the Kuroshio Extension front in the early summer of 2012: three-vessel simultaneous observations and numerical simulations. J. Oceanogr., 71, 511–526, doi:10.1007/s10872-014-0266-0.
Konda, M., H. Ichikawa, H. Tomita, and M. F. Cronin, 2010: Surface heat flux variations across the Kuroshio Extension as observed by surface flux buoys. J. Climate, 23, 5206–5221, doi:10.1175/2010JCLI3391.1.
Kwon, Y.-O., M. A. Alexander, N. A. Bond, C. Frankignoul, H. Nakamura, B. Qiu, L. A. Thompson 2010: Role of the Gulf Stream and Kuroshio–Oyashio Systems in large-scale atmosphere–ocean interaction: A review, J. Climate, 23, 3249-3281, doi: 10.1175/2010JCLI3343.1.
Legg, S., and J. C. McWilliams, 2002: Sampling characteristics from isobaric floats in a convective eddy field. J. Phys. Oceanogr., 32, 527–534, doi:10.1175/1520-0485.
Lindstrom, E. and Coauthors, 2012: A framework for ocean observing. UNESCO, IOC-INF-1284, doi:10.5270/OceanObs09-FOO
Ma, X., P. Chang, R. Saravanan, R. Montuoro, J.-S. Hsieh, D. Wu, X. Lin, L. Wu, and Z. Jing, 2015: Distant influence of Kuroshio Eddies on North Pacific weather patterns? Scient. Rep., 5, doi:10.1038/srep17785
Ma, X., and Coauthors, 2016: Western boundary currents regulated by interaction between ocean eddies and the atmosphere. Nature, 535, 533–537. doi:10.1038/nature18640
Mahadevan, A. 2016: The impact of submesoscale physics on primary productivity of plankton. Ann. Rev. Mar. Sci., 8, 161-184, doi: 10.1146/annurev-marine-010814-015912.
McGillicuddy, D. J. 2016: Mechanisms of physical-biological-biogeochemical interaction at the oceanic mesoscale. Ann. Rev. Mar. Sci., 8, 125-159, doi: 10.1146/annurev-marine-010814-015606.
Meinig, C., N. Lawrence-Slavas, R. Jenkins, and H.M. Tabisola. 2015: The use of Saildrones to examine spring conditions in the Bering Sea: Vehicle specification and mission performance. OCEANS 2015 – MTS/IEEE Washington. October 19–22, 2015, Washington, DC, 1-6, doi: 10.23919/OCEANS.2015.7404348.
Minobe, S., A. Kuwano-Yoshida, N. Komori, S. P. Xie, and J. R. Small, 2008: Influence of the Gulf Stream on the troposphere. Nature, 452, 206–209, doi:10.1038/nature06690.
Mordy, C. W., and Coauthors, 2017: Advances in ecosystem research: Saildrone surveys of oceanography, fish, and marine mammals in the Bering Sea. Oceanogr., 30, 113–115, doi: 10.5670/oceanog.2017.230.
Nagai, T., and S. Clayton, 2017: Nutrient interleaving below the mixed layer of the Kuroshio Extension Front. Ocean Dyn., 67, 1027-1046, doi:10.1007/s10236-017-1070-3.
Nagano, A., T. Suga, Y. Kawai, M. Wakita, K. Uehara, and K. Taniguchi, 2016: Ventilation revealed by the observation of dissolved oxygen concentration south of the Kuroshio Extension during 2012–2013. J. Oceanogr., 72, 837–850, doi:10.1007/s10872-016-0386-9.
Nakamura, H., A. Isobe, S. Minobe, H. Mitsudera, M. Nonaka, and T. Suga, 2015: “Hot Spots” in the climate system—new developments in the extratropical ocean–atmosphere interaction research: a short review and an introduction. J. Oceanogr., 71, 463–467, doi:10.1007/s10872-015-0321-5.
Oka, E., B. Qiu, Y. Takatani, K. Enyo, D. Sasano, N. Kosugi, M. Ishii, T. Nakano, and T. Suga, 2015: Decadal variability of subtropical mode water subduction and its impact on biogeochemistry. J. Oceanogr., 71, 389-400, doi: 10.1007/s10872-015-0300-x.
Palevsky, H. I., Quay, P. D., Lockwood, D. E., & Nicholson, D. P. 2016: The annual cycle of gross primary production, net community production, and export efficiency across the North Pacific Ocean. Glob. Biogeochem. Cycles, 30, doi:10.1002/2015GB005318
Qiu, B., P. Hacker, S. Chen, K. A. Donohue, D. R. Watts, H. Mitsudera, N. G. Hogg and S. R. Jayne, 2006: Observations of the subtropical mode water evolution from the Kuroshio Extension System Study. J. Phys. Oceanogr., 36, 457-473, doi:10.1175/JPO2849.1.
Rainville, L., S. R. Jayne, and M. F. Cronin, 2014: Variations of the North Pacific subtropical mode water from direct observations. J. Climate, 27, 2842-2860, doi:10.1175/JCLI-D-13-00227.1.
Rio, M.-H., and F. Hernandez, 2004: A mean dynamic topography computed over the World Ocean from altimetry, in situ measurements, and a geoid model. J. Geophys. Res., 109, doi:10.1029/2003JC002226.
Sabine, C. L., Feely, R. A., Gruber, N., Key, R. M., Lee, K., Bullister, J. L., Wanninkhof, R., Wong, C., Wallace, D. W. R., Rilbrook, B., Millero, F. J., Peng, T.-H., Kozyr, A., Ono, T., and Rios, A. F., 2004. The oceanic sink for anthropogenic CO2. Science, 305, 367–371, doi:10.1126/science.1097403.
Shcherbina, A. Y., and Coauthors, 2014: The LatMix Summer Campaign: Submesoscale stirring in the upper ocean. Bull. Amer. Meteorol. Soc., 96, 1257–1279, doi:10.1175/BAMS-D-14-00015.1
Small, R. J., S. P. deSzoeke, S. P., Xie, L. O’Neill, H. Seo, Q. Song, Q., P. Cornillon, M. Spall, and S. Minobe, 2008: Air-sea interaction over ocean fronts and eddies. Dyn. Atmos. Oceans, 45, 274–319, doi:10.1016/j.dynatmoce.2008.01.001.
Smith, S. R., N. Lopez, and M. A. Bourassa, 2016: SAMOS air-sea fluxes: 2005–2014. Geosci. Data J., 3, 9-19, doi: 10.1002/gdj3.34.
Takahashi, T., and Coauthors, 2009: Climatological mean and decadal change in surface ocean pCO2, and net sea–air CO2 flux over the global oceans. Deep-Sea Res. Part II: Top. Stud. Oceanogr., 56, 554–577, doi:10.1016/j.dsr2.2008.12.009.
Trenberth, K. E., and A. Solomon 1994: The global heat balance: Heat transports in the atmosphere and ocean, Climate Dyn., 10, 107–134, doi: 10.1007/BF00210625.
Thomas, L. N., J. R. Taylor, E. D’Asaro, C. M. Lee, J. M. Klymak, and A. Shcherbina, 2015: Symmetric instability, inertial oscillations, and turbulence at the Gulf Stream front. J. Phys. Oceanogr., 46, 197–217, doi:10.1175/JPO-D-15-0008.1.
Thomson, J., and J. Girton, 2017: Sustained measurements of Southern Ocean air-sea coupling from a Wave Glider autonomous surface vehicle. Oceanogr., 30, 104–109, doi: 10.5670/oceanog.2017.228.
Wakita, M., and Coauthors, 2016: Biological organic carbon export estimated from the annual carbon budget observed in the surface waters of the western subarctic and subtropical North Pacific Ocean from 2004 to 2013. J. Oceanogr, 72, 1–21. doi:10.1007/s10872-016-0379-8.
Xu, L., P. Li, S.-P. Xie, Q. Liu, Q., C. Liu, and W. Gao, 2016: Observing mesoscale eddy effects on mode-water subduction and transport in the North Pacific. Nature Comm., 7, doi:10.1038/ncomms10505.
Yu, L. and R. A. Weller, 2007: Objectively analyzed air-sea heat fluxes for the global ice-free oceans (1981–2005). Bull. Amer. Meteor. Soc., 88, 527–539, doi: 10.1175/BAMS-88-4-527.
Zhang, D., W. E. Johns, and T. N. Lee 2002: The seasonal cycle of meridional heat transport at 24°N in the North Pacific and in the global ocean. J. Geophys. Res.: Oceans, 107, 1-24, doi:10.1029/2001JC001011