Alison R. Gray1, Jaime Palter2
1. University of Washington
2. University of Rhode Island
Estimates of contemporary global air-sea carbon dioxide (CO2) flux (Takahashi et al. 2009; Landschützer et al. 2014) suggest that subtropical western boundary currents (WBCs) and their zonal extensions are key regions of oceanic carbon uptake (Figure 1a). These narrow, intensified currents, which transport water poleward along the western edge of every ocean basin before separating from the continental shelf and turning eastward, are associated with maxima in mean velocity, eddy kinetic energy, air-sea heat flux, and nutrient transport, as well as deep mixed layers on their equatorward flanks. The prominence of these regions in the global air-sea flux of CO2 leads to a basic question: What is the role of the WBCs and their zonal extensions in the global carbon cycle? The answer, which involves the physics and biogeochemistry of the ocean-atmosphere system across a wide range of temporal and spatial scales, reveals the complex nature of these systems and their importance within the climate system, while also highlighting a number of the gaps in our current understanding.
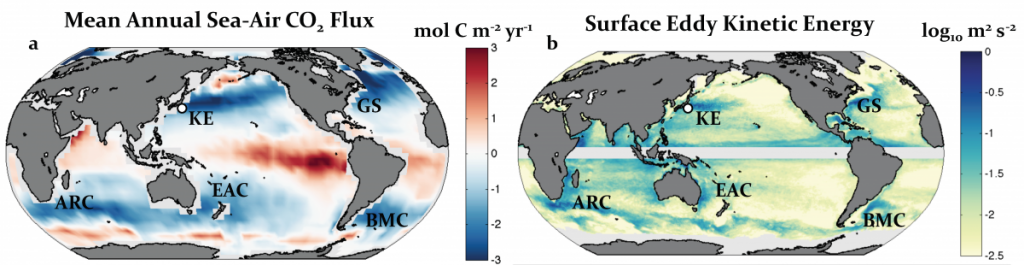
Figure 1: (a) Climatological mean annual sea-air CO2 flux referenced to the year 2000 adapted from Takahashi et al. 2009. Blue (red) areas are ocean sink (source) regions for atmospheric CO2. (b) Surface eddy kinetic energy calculated from the 2011-2013 daily AVISO sea surface height product. The white circle in a and b indicates the location of the Kuroshio Extension Observatory mooring. WBC systems are labeled as follows: Kuroshio Extension (KE), Gulf Stream (GS), Agulhas Return Current (ARC), East Australian Current (EAC), and Brazil-Malvinas Confluence (BMC). Courtesy A. Fassbender and S. Bishop.
Air-sea carbon fluxes are primarily controlled by the difference between the partial pressure of CO2 in the atmosphere, which has been steadily increasing since the 1870s due to the burning of fossil fuels, and the partial pressure of CO2 in seawater (pCO2), which can be influenced by many factors (Sarmiento and Gruber 2006). The contemporary oceanic carbon uptake in the subtropical WBCs and their extensions comes about from both thermally and biologically driven decreases in pCO2. The first of these is tied to the role that WBCs play in the large-scale circulation of the ocean. These currents supply the poleward return flow for the large-scale wind-driven circulation that, through Sverdrup dynamics, generates upper ocean equatorward flow across the vast subtropical gyres (Vallis 2006). As a result, the vigorous (on the order of 1 m s-1) flows in the WBCs rapidly transport warm water from the tropics to mid-latitudes. At mid-latitudes in winter, the atmospheric storm track advects frigid air across the continents and eastward over these warm currents, leading to the largest air-sea heat fluxes in the global ocean. This physical phenomenon has a direct impact on air-sea carbon flux through the solubility effect, whereby ocean cooling reduces pCO2 and thus drives the system towards more uptake by the ocean. The second mechanism for lowering surface ocean pCO2 and producing a flux into the ocean is biological production of organic matter in the euphotic zone, which also occurs at high rates in and around WBCs and their extension regions. This biological productivity is supported by deep convective mixing on the equatorward fringes of the WBCs, which brings nutrients up from the seasonal thermocline, as well as the cross-WBC transport of nutrients. Together these two factors ¾ solubility effects and primary productivity ¾ act to decrease pCO2 in WBCs and their extensions, creating hotspots of ocean carbon uptake.
The impact of air-sea CO2 fluxes on the global climate system hinges on the fate of carbon after it enters the surface ocean, and in this regard, the WBC regions also play a crucial role. The significant heat loss to the atmosphere and strong winds that combine to create substantial solubility-driven carbon uptake also lead to some of the deepest wintertime mixed layers in the global ocean on the equatorward side of the WBCs. These thick mixed layers are subsequently capped by lighter waters during springtime restratification and then subducted into the ocean interior to form the subtropical mode waters (STMWs) found in each basin. The carbon contained in these mode waters is thus isolated from the atmosphere. How, when, and where STMWs subsequently re-enter the ocean mixed layer governs the influence of WBC carbon uptake on atmospheric carbon concentrations and helps determine the future evolution of the ocean carbon sink (Gruber et al. 2002). Alternatively, carbon taken up in the surface ocean can enter the interior ocean via sinking organic matter that is then remineralized. Depending on the depths to which biological particles sink, this carbon can be trapped below the surface for potentially much longer timescales.
In addition to the role that STMWs play in the transfer of carbon from the surface ocean to the thermocline, the process of mode water formation has several other effects on the oceanic carbon cycle. The deepening of the mixed layer entrains subsurface waters that are higher in dissolved inorganic carbon and nutrients. The resulting increase in mixed layer carbon acts to reduce the pCO2 gradient across the air-sea interface, dampening ocean carbon uptake. The increase in nutrients, however, can also stimulate phytoplankton growth, leading to more biologically driven CO2 uptake. The balance between these processes, which can vary enormously in space and time, will regulate the total carbon uptake and storage in STMWs. If inorganic carbon and nutrients are entrained into the mixed layer at the same ratio as is removed in sinking organic particles, then primary production will not have a net impact on oceanic CO2 uptake. However, the physical processes that govern both mixed layer dynamics and the upper ocean circulation, as well as the biological processes that determine productivity and export, are frequently decoupled in both space and time. Accordingly, variability in biologically driven carbon uptake can exist on timescales of seasons to decades or longer.
All of the processes described thus far are believed to have been operating since well before anthropogenic perturbations to the atmospheric CO2 concentration. Reconstructions of atmospheric CO2 from ice cores suggest that, averaged over decades, Holocene concentrations were near steady state (Ciais et al. 2013). Therefore, the carbon taken up by the ocean in WBC regions was balanced by outgassing elsewhere, in the climatological mean. The carbon that moves through the climate system through this pre-industrial carbon cycle, referred to as natural carbon, is often conceptually separated from the anthropogenic carbon added to the atmosphere by fossil fuel burning, of which approximately 30% has been absorbed by the ocean (Le Quéré et al. 2016). Modeling and observational studies point to WBCs as being important regions for uptake of anthropogenic carbon, and the STMWs formed on the equatorward flanks of the WBCs account for a significant portion of the anthropogenic carbon storage (Sabine et al. 2004; Iudicone et al., 2016). Climate model-based projections indicate that WBCs and their extensions will continue to be important sinks of carbon as the climate warms (McKinley et al. 2017). However, from observations it is quite difficult to disentangle the natural carbon cycle from the anthropogenic perturbation, and thus natural variability may significantly affect the rates of carbon uptake and storage that we observe.
Together, solubility and biological effects as well as anthropogenic carbon uptake act in concert to create the hotspots of ocean carbon uptake in the WBCs that we observe today. As we become progressively better able to observe and model the climate system, with longer observational records and greater resolution in both space and time, the more variability we find in these highly dynamic regions of the global ocean. WBCs are notable for significant energy at the mesoscale level, i.e., motions at the scales of approximately one month and 100 km at the WBC latitudes, as evidenced by the mean variability in the altimetric sea surface height (Figure 1b). Intense eddying occurs here because WBCs are regions of strong fronts and thus steeply tilted isopycnals. The significant potential energy inherent in this condition leads to the growth of baroclinic instabilities and the substantial mesoscale variability observed in the WBCs.
Mesoscale features can impact the oceanic carbon cycle in a number of different ways. Mesoscale motions strongly affect mixed layer depths, with implications for mode water formation, subduction, and ventilation; the net effect is to increase the stratification of the upper ocean. Many studies have shown that mesoscale eddies, in releasing the potential energy associated with tilted isopycnals, pump heat out of the deep ocean and towards the surface (Gregory 2000; Gnanadesikan et al. 2005; Palter et al. 2014). Given that natural carbon increases with depth in the ocean due to the remineralization of sinking organic matter, this eddy-driven vertical exchange is expected to reduce the strength of the biological pump. On the other hand, anthropogenic carbon concentrations are highest at the ocean’s surface and decrease downward, so that the global-average effect of mesoscale eddies would be expected to decrease the ocean uptake of anthropogenic CO2, relative to an ocean without such eddies. To infer the current generation of climate models’ ability to represent the net effect of eddies on carbon through common parameterizations, we take cues from an analysis of ocean heat uptake in models of varying resolution (Griffies et al. 2015): Parameterizations were successful in their qualitative representation of the upward transport of heat, albeit at a reduced efficiency relative to the simulations that resolved a rich spectrum of mesoscale eddies. This conclusion suggests that, in the model analyzed, parameterized eddies might underestimate the upward pumping of natural carbon from the ocean interior and overestimate the unbalanced downward transport of anthropogenic carbon by the time-mean circulation.
As a result of the significant horizontal and vertical shear associated with the high levels of mesoscale activity found in the WBCs, these regions are also hotspots of variability at the submesoscales, or motions at scales of approximately 10 km and one day. Such motions, which can be generated through a number of different mechanisms, can be associated with substantial instantaneous vertical velocities and are thought to be critical in the restratification of the mixed layer. Submesoscale motions can lead to strong physical export of particles (Omand et al. 2014), and their effects are only partially parameterized in current generation of climate models. While a number of ambitious field campaigns have been conducted in recent years in order to better understand these types of motions (e.g., Shcherbina et al. 2015), there are still many open questions regarding the impact of small-scale instabilities on the uptake and storage of carbon through both biophysical coupling and effects on mode water subduction and ventilation.
Diverse physical and biological processes, at a range of temporal and spatial scales, clearly contribute to making the WBCs hotspots of oceanic carbon uptake. While the large-scale mean state of the system is relatively well-understood, a number of challenges remain that currently limit our understanding of the role that WBCs and their extensions play in the global carbon cycle. The spatial and temporal variability in the natural carbon cycle of these systems is not well-characterized, due to the difficulties inherent in both observing and modeling these extremely turbulent regions. Given that the WBC uptake of total carbon (the sum of the natural and anthropogenic components) is determined by the balance of large fluxes both into and out of the ocean, variability in the processes that govern these fluxes can have important effects on the ocean carbon cycle. Although our knowledge of mesoscale and submesoscale physics has increased immensely over the past few decades, how these motions impact biogeochemical cycling at small scales and how these effects feed back on the larger-scale carbon uptake and storage are still open questions. In addition, the Southern Hemisphere WBCs are generally much less studied than their counterparts in the North Atlantic and North Pacific basins, despite the fact that these systems connect to and interact with the Southern Ocean where model estimates indicate approximately 50% of the ocean uptake of anthropogenic carbon has occurred (Frölicher et al. 2015). Indeed, one of the biggest bottlenecks in both quantifying and understanding the role of WBCs in the global carbon cycle remains the chronic scarcity of observations in the Southern Hemisphere.
As we work toward addressing these issues, it remains critically important to develop a mechanistic understanding of the myriad processes that are involved in carbon uptake in the WBCs and their extensions. In this way, we will be able to translate this knowledge into better predictions of future changes in the role of WBCs in the global carbon cycle.
References
Ciais, P., and Coauthors, 2013: Carbon and Other Biogeochemical Cycles. Climate Change 2013: The Physical Science Basis. Contribution of Working Group I to the Fifth Assessment Report of the Intergovernmental Panel on Climate Change, T. F. Stocker, D. Qin, G.-K. Plattner, M. Tignor, S. K. Allen, J. Boschung, A. Nauels, Y. Xia, V. Bex, and P. M. Midgley, Eds., Cambridge University Press, Cambridge, United Kingdom, and New York, USA, doi:10.1017/CBO9781107415324.015.
Frölicher, T. L., J. L. Sarmiento, D. J. Paynter, J. P. Dunne, J. P. Krasting, and M. Winton, 2015: Dominance of the Southern Ocean in anthropogenic carbon and heat uptake in CMIP5 models. J. Climate, 28, 862–886, doi:10.1175/JCLI-D-14-00117.1
Gnanadesikan, A., R. D. Slater, P. S. Swathi, and G. K. Vallis, 2005: The energetics of ocean heat transport, J. Climate, 18, 2604–2616, doi:10.1175/jcli3436.1.
Gregory, J. M., 2000: Vertical heat transports in the ocean and their effect on time-dependent climate change. Climate Dyn., 16, 501-515, doi:10.1007/s003820000059.
Griffies, S. M. and Coauthors, 2015: Impacts on ocean heat from transient mesoscale eddies in a hierarchy of climate models. J. Climate, 28, 952–977, doi:10.1175/JCLI-D-14-00353.1.
Gruber, N., C. D. Keeling, and N. R. Bates, 2002: Interannual variability in the North Atlantic ocean carbon sink. Science, 298, 2374–2378, doi:10.1126/science.1077077.
Iudicone, D., and Coauthors, 2016: The formation of the ocean’s anthropogenic carbon reservoir. Scientific Reports, 6, 35473. http://doi.org/10.1038/srep35473
Landschützer, P., N. Gruber, D. C. E. Bakker, and U. Schuster, 2014: Recent variability of the global ocean carbon sink. Global Biogeochemical Cycles, 28, 927–949. http://doi.org/10.1002/2014GB004853
Le Quéré, C., and Coauthors, 2016: Global Carbon Budget 2016. Earth Syst. Sci. Data., 8, 605-649. DOI:10.5194/essd-8-605-2016.
McKinley, G. A., A. R. Fay, N. S. Lovenduski, and D. J. Pilcher, 2017: Natural variability and anthropogenic trends in the ocean carbon sink. Annu. Rev. Mar. Sci., 9, 125–50. http://doi.org/10.1146/annurev-marine-010816-060529
Omand, M. M., E. A. D’Asaro, C. M. Lee, M. J. Perry, N. Briggs, I. Cetini, and A. Mahadevan, 2015: Eddy-driven subduction exports particulate organic carbon from the spring bloom. Science, 348, 222–225. http://doi.org/10.1126/science.1260062
Palter, J. B., S. M. Griffies, B. L. Samuels, E. D. Galbraith, A. Gnanadesikan, and A. Klocker, 2014: The deep ocean buoyancy budget and its temporal variability. J. Climate, 27, 551–573, doi:10.1175/JCLI-D-13-00016.1.
Sabine, C. L., and Coauthors, 2004: The oceanic sink for anthropogenic CO2. Science, 305, 367–371. http://doi.org/10.1126/science.1097403
Sarmiento, J. L., and N. Gruber, 2006: Ocean Biogeochemical Dynamics. Princeton University Press, New Jersey, USA, ISBN:9781400849079.
Shcherbina, A. Y., and Coauthors, 2015: The LatMix summer campaign: Submesoscale stirring in the upper ocean. Bulletin of the American Meteorological Society, 96, 1257–1279. http://doi.org/10.1175/BAMS-D-14-00015.1
Takahashi, T., and Coauthors, 2009: Climatological mean and decadal change in surface ocean pCO2, and net sea–air CO2 flux over the global oceans. Deep-Sea Res. Part II: Top. Stud. Oceanogr., 56, 554–577, doi:10.1016/j.dsr2.2008.12.009.
Vallis, G. K., 2006: Atmospheric and Oceanic Fluid Dynamics: Fundamentals and Large-Scale Circulation. Cambridge University Press, Cambridge, UK, ISBN: 9780521849692.