The El Niño-Southern Oscillation (ENSO) is the dominant mode of tropical Pacific climate variability at interannual timescales, with profound influences on seasonal weather and ecosystems worldwide. In particular, the physical and biological conditions along the US West Coast, an area that supports one of the most productive marine ecosystems in the world, are strongly influenced by ENSO. Specifically, during El Niño events, alongshore winds weaken and upwelling is reduced, resulting in warmer surface waters, reduced nutrient supply to the euphotic zone, and reduced biological productivity. While these conditions during El Niño events are well known, the exact mechanisms involved and the origin of event-to-event differences in ENSO impacts are not fully understood. Here, we review our current state of knowledge on ENSO and its different expressions, the mechanisms by which ENSO influences the US West Coast, and possible approaches for understanding the predictability of those impacts.
ENSO dynamics and oceanic teleconnections
Tropical Pacific interannual variations involve changes in the thermocline, namely the interface between the warmer upper ocean layer and the colder deeper ocean. In its neutral state, the tropical Pacific is characterized by a shallower thermocline in the eastern Pacific and deeper thermocline in the western Pacific, with a zonal (east-west) slope that is in equilibrium with the surface easterly wind stress. Surface waters are thus colder in the eastern Pacific “Cold Tongue,” and much warmer west of the dateline in the western Pacific “Warm Pool.” ENSO events are disruptions of this neutral state. During warm events, the El Niño phase, the easterly trades weaken, reducing upwelling in the Cold Tongue region. The thermocline deepens in the east and shoals in the west (Figure 1) and the zonal temperature gradient is reduced. The initial deepening of the eastern Pacific thermocline is achieved through the eastward propagation of downwelling Kelvin waves, excited by high-frequency winds in the form of westerly wind events (WWEs) in the western Pacific (McPhaden 1999, Roundy and Kiladis 2006), and amplified by slower-building wind anomalies (known as the Bjerknes feedback). After reaching the eastern ocean boundary, these Kelvin waves continue poleward along the coastlines of the Americas as coastally trapped Kelvin waves, depressing the thermocline, and reducing upwelling along the west coast of North and South America. The coastal wave propagation north of the Equator can clearly be seen in Figure 1 all the way to Baja California. In contrast, upwelling Kelvin waves during La Niña conditions induce a shoaling of the thermocline in the eastern equatorial Pacific and along the west coast of the Americas, resulting in increased upwelling (Simpson 1984; Lynn and Bograd 2002; Huyer et al. 2002; Bograd et al. 2009; Hermann et al. 2009; Miller et al. 2015).
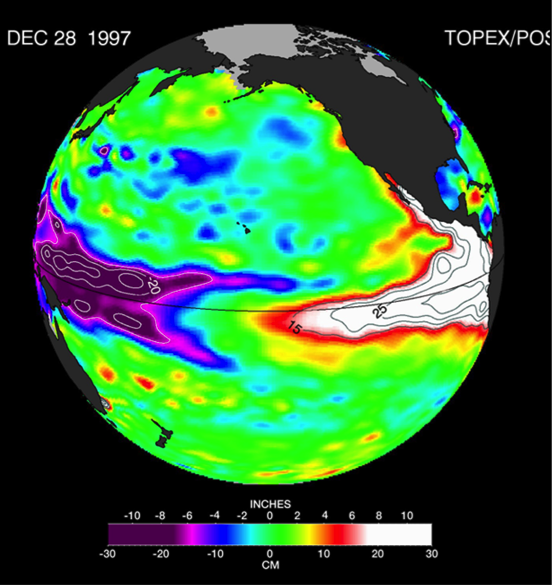
Figure 1. Canonical oceanic teleconnection pattern associated with coastally trapped Kelvin waves emanating from the tropical and subtropical eastern Pacific during the 1997-98 El Niño, as revealed by sea surface height altimeter observations (Credit: NASA/JPL-Caltech). These boundary-trapped waves have the potential to travel from the Equatorial region to the California Coast (and beyond) where they can alter thermocline depth, SST, mixed-layer depth, and currents. Atmospheric teleconnections, however, can also drive regional oceanic anomalies that mimic this same type of response.
The changes in upwelling associated with the coastal Kelvin waves can directly impact the biogeochemistry of the waters along the US West Coast. However, the offshore scale of the waves decreases with latitude, and the waves decay while propagating northward due to dissipation and radiation of energy by the generation of westward propagating Rossby waves (Marchesiello et al. 2003). In addition, topography and bathymetry can modify the nature of the waves and perhaps partially impede their propagation at some locations, casting some doubt on the effectiveness of coastal waves of equatorial origin to substantially alter the stratification along the US West Coast and modulate the local marine ecosystem.
Atmospheric teleconnections
Equatorial sea surface temperature (SST) anomalies associated with ENSO also influence remote weather and climate through large-scale atmospheric teleconnections. Variations in convection trigger atmospheric stationary Rossby wave trains that alter the Pacific North America Pattern (PNA, Figure 2), a mode of North Pacific geopotential height variability (Horel and Wallace 1981), and induce variations in the regional atmospheric circulation. In particular, El Niño events are associated with an intensification and southward shift of the Aleutian Low (AL) pressure system and changes in the eastern Pacific subtropical high, which conspire to weaken the alongshore winds off the US West Coast, resulting in reduced upwelling and warmer SST. These changes associated with the local atmospheric forcing are similar to those induced by coastal Kelvin waves of equatorial origin, making it very difficult to distinguish the relative importance of the oceanic and atmospheric pathways in this region, especially observationally. In addition, large uncertainties exist surrounding the atmospheric mid-latitude response to tropical SST anomalies. Results from a recent study based on both observations and climate model ensemble simulations indicate that uncertainties in the sea level pressure (SLP) response to ENSO arise primarily from atmospheric internal variability rather than diversity in ENSO events (Deser et al. 2017). Thus, the details of the ENSO teleconnections can vary significantly and randomly from event to event and result in important differences along the California Coast.
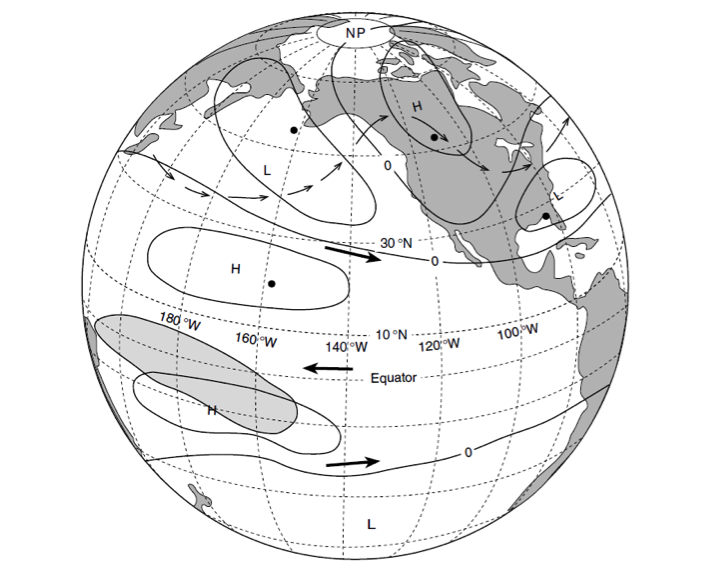
Figure 2. Canonical wintertime atmospheric teleconnection pattern associated with ENSO as a response to tropical heating, also known as the Pacific North American (PNA) pattern, as schematically illustrated by Horel and Wallace (1981). The contour lines represent middle troposphere geopotential height anomalies that occur in response to warm SST in the tropical Pacific near the dateline during an El Niño (shaded area). The Rossby wave-like pattern includes high-pressure anomalies in the Northern Hemispheric subtropics and low-pressure anomalies in the North Pacific, with a ridge over Canada and an anomalous low-pressure region in the Southeastern US. The dark arrows depict the strengthened subtropical jets and easterlies near the dateline. The lighter arrows indicate the distorted mid-tropospheric streamlines due to troughing and ridging.
ENSO diversity and its implications for impacts on the US West Coast
As already noted by Wyrtki (1975), “No two El Niño events are quite alike.” Indeed, ENSO events differ in amplitude, duration, and spatial pattern, and several studies have suggested that such differences may play an important role in ENSO impacts (see Capotondi et al. 2015 for a review). Special emphasis has been given to the location of the maximum equatorial SST anomalies, as this is an aspect that is readily observed and may influence atmospheric teleconnections (Ashok et al. 2007; Larkin and Harrison 2005). Although the longitudinal position of the maximum SST anomalies along the equator varies from event to event in a quasi-continuum fashion, for practical purposes, events are often grouped depending on whether the largest anomalies are located in the eastern Pacific (“EP” events), or in the central Pacific (“CP” events). Here, we use the relative amplitudes of SST anomalies in the Niño-3 (5°S-5°N, 150°W-90°W) and Niño-4 (5°S-5°N, 160°E-150°W) regions to classify the events as “EP” or “CP”. Figure 3 shows the equatorial profiles of SST anomalies for the two groups of events in the Simple Ocean Data Assimilation (SODA; Carton and Giese 2008) reanalysis over the period 1958-2007 (Figure 3a) and in 500 years of a pre-industrial control simulation of the National Center for Atmospheric Research (NCAR) Community Climate System Model version 4 (CCSM4; Figure 3b). We notice that there is a large overlap between the two groups of events, which is indicative of the large spread in event longitudinal distribution, although events peaking in the eastern Pacific can achieve larger amplitudes than those peaking in the central Pacific. This difference in amplitude is not as pronounced in the precipitation profiles (Figure 3c), suggesting that in spite of their weaker SST anomaly signature, CP events may still have a large influence on the atmosphere due to their position in a region of warmer background SST.
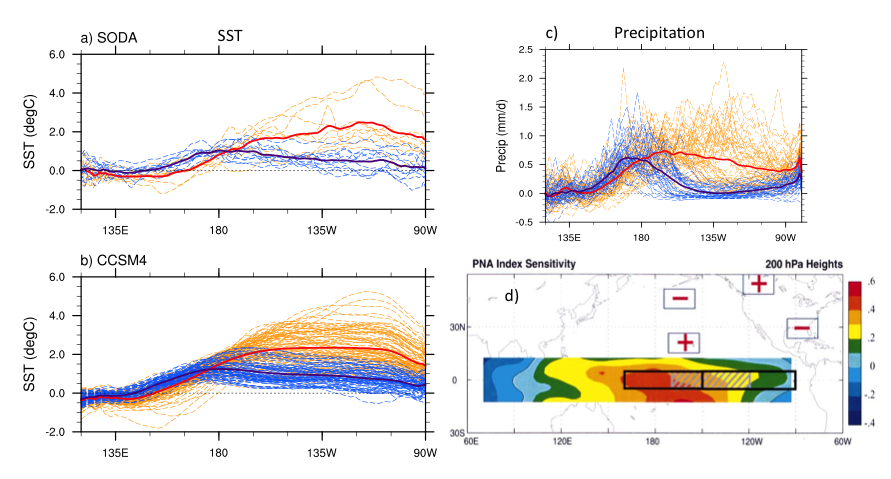
Figure 3. a) Equatorial SST anomaly profiles for El Niño events with largest SST anomalies in the Niño-3 region (EP events, thin dashed orange lines) and in the Niño-4 region (CP events, thin dashed blue lines) from the SODA ocean reanalysis over the period 1958-2007. The thick red and blue lines are the composites of the thin orange and blue lines, respectively. b) Same as in a, but for a 500-year preindustrial simulation of the NCAR-CCSM4 climate model. c) Same as in b, but for precipitation anomalies rather than SST anomalies. The a), b) and c) panels are adapted from Capotondi (2013). d) Tropical SST anomaly pattern, or “sensitivity pattern,” that exerts the largest influence on the PNA (the “+” and “-“ signs indicate the PNA highs and lows as shown in Figure 2), as computed by Barsugli and Sardeshmukh (2002) using ensembles of atmospheric model simulations forced by a set of SST anomaly patches over the tropical Pacific. Panel c) is adapted from Barsugli and Sardeshmukh (2002).
Do different types of ENSO events have different impacts on the climate and marine ecosystems of the US West Coast? In terms of atmospheric teleconnections, “canonical” EP events have been associated with changes in the AL, while CP events may produce a strengthening of the second mode of North Pacific atmospheric variability, the North Pacific Oscillation (NPO; Di Lorenzo et al. 2013). AL variability is associated with the Pacific Decadal Oscillation, while the NPO appears to provide the atmospheric forcing for the North Pacific Gyre Oscillation (Di Lorenzo et al. 2008), a mode of variability that is largely correlated with biologically relevant quantities along the West Coast of the US. However, the event-to-event differences in teleconnections, associated with intrinsic atmospheric variability, may obscure differences in atmospheric response to different event types.
EP and CP events have different subsurface characteristics as well so that the oceanic pathways between the tropical Pacific and the US West Coast can also be expected to differ in the two cases. While EP events are characterized by large equatorial thermocline anomalies across the basin, which evolve consistently with the recharge oscillator paradigm (Jin 1997), thermocline depth anomalies during CP events tend to be confined to the central part of the basin and do not undergo the large variations associated with the meridional warm water volume transport. As a result, the Kelvin wave signature in the eastern equatorial Pacific, and the resulting amplitude of the coastal Kelvin wave can be expected to be weaker during CP events. Indeed, a recent study (Fischer et al. 2015) has shown that temperature anomalies (and associated zooplankton composition) in the northern California Current responded very rapidly to EP El Niño events with a peak during boreal winter, whereas CP events were accompanied by a delayed response with a peak during boreal spring. The most recent 2015/16 El Niño provides another compelling example of diversity in ENSO influences. In spite of the magnitude of the event, which was comparable to the previous two extreme events on record, the 1982/83 and 1997/98, the changes in temperature, thermocline/nutricline depth, and alongshore winds associated with this event were much smaller than during the two previous cases (Jacox et al. 2016). These differences are perhaps due to the unique nature of this event, whose spatial pattern has elements of both EP and CP El Niño types, with, in particular, a weaker thermocline depth anomaly in the eastern equatorial Pacific relative to the 1982/83 and 1997/98 cases. This question remains open and is the subject of intense research.
How well can we predict different types of ENSO events? Several studies have attempted to determine specific precursors for EP- and CP-type events. SST and wind stress anomalies propagating southwestward from the Southern California coast to the central equatorial Pacific, a pattern known as the “Pacific Meridional Mode” (PMM; Chiang and Vimont 2004) has been suggested as a possible precursor for CP events (Yu and Kim 2011; Vimont et al. 2014), while SST and wind stress anomalies extending northward along the coast of South America toward the eastern equatorial Pacific (the “South Pacific Meridional Mode” or SPMM; Zhang et al. 2014) have been considered as candidate precursors for EP-type events. While these modes of variability do produce initial SST anomalies either in the central or eastern Pacific, these anomalies can propagate along the equator and maximize at a different longitude in the mature phase of the event. For example, the strong 1982/83 EP El Niño developed from anomalous SSTs in the central Pacific in the late spring of 1982, which propagated eastward to achieve their largest amplitude near the South American coast in the following winter (Xue and Kumar 2016). In late spring 2015, on the other hand, anomalies exceeding 2°C appeared in the far eastern Pacific and then propagated westward to reach their largest amplitude in the central Pacific in winter (Xue and Kumar 2016). While several studies have emphasized SST precursors, thermocline conditions two seasons prior to the peak of an event appear to play an important role in the development of the two types of events (Capotondi and Sardeshmukh 2015). Deeper than average initial thermocline conditions in the eastern Pacific favor EP-type events and shallower than average eastern Pacific thermocline depth favors CP-type events. The results of Capotondi and Sardeshmukh (2015) were obtained using a combination of multiple linear regressions and linear inverse modeling (Penland and Sardeshmukh 1995), thus objectively providing the initial state that will optimally evolve, two seasons later, in either an EP- or CP-type event.
Given the remaining uncertainties in the exact triggers of ENSO diversity, as well as the large noise level of atmospheric teleconnections, how can we isolate the predictable component of the ENSO influence on the US West Coast physical and biogeochemical conditions in the Pacific? In other words, even if we could perfectly predict ENSO in all its diversity and atmospheric teleconnections, how well could we predict the ecosystem responses? One possible approach is to determine the SST pattern to which a given target quantity (e.g., a mode of atmospheric variability or some local ecosystem forcing function) is most sensitive. The SST anomalies that are most effective in influencing specific “target” regions do not necessarily coincide with the anomalies typical of “canonical” ENSO events (Rasmussen and Carpenter 1982). In fact, as shown by Barsugli and Sardeshmukh (2002) the PNA pattern is particularly sensitive to SST anomalies in the Niño-4 region rather than the Niño-3 region where canonical “EP” events typically peak (Figure 3d). This implies that weaker CP El Niño events may exert a comparable influence on the sensitivity pattern relative to stronger EP events, and be as (if not more) effective in influencing atmospheric teleconnections like the PNA (compare Figures 3a,b with Figure 3d). Similar sensitivity patterns could be determined for key regional forcing function along the US West Coast, either using the approach outlined in Barsugli and Sardeshmukh (2002) or via multiple linear regression (e.g., Capotondi and Sardeshmukh 2015).
Conclusions
In summary, ENSO can provide a large source of potential predictability for the physics and the biology of the US West Coast. However, in light of the large uncertainties associated with ENSO diversity and atmospheric teleconnections, novel approaches need to be developed to isolate the robust predictable components of ENSO influences and inform forecast development.
Authors
Antonietta Capotondi (NOAA Earth System Research Laboratory)
Kris Karnauskas (University of Colorado, Boulder)
Arthur Miller (Scripps Institution of Oceanography)
Aneesh Subramanian (University of Oxford, UK)
References
Ashok, K., S. K. Behera, S. A. Rao, H. Weng, and T. Yamagata, 2007: El Niño Modoki and its possible teleconnections. J. Geophys. Res., 112, doi:10.1029/2006JC003798.
Barsugli, J. J., and P. D. Sardeshmukh, 2002: Global atmospheric sensitivity to tropical SST anomalies throughout the Indo-Pacific basin. J. Climate, 15, 3427-3442, doi: 10.1175/1520-0442(2002)015<3427:GASTTS>2.0.CO;2.
Bograd, S. J., I. Schroeder, N. Sarkar, X. Qiu, W. J. Sydeman, and F. B. Schwing, 2009: Phenology of coastal upwelling in the California Current. Geophys. Res. Lett., 36, doi: 10.1029/2008GL035933.
Capotondi, A., 2013: ENSO diversity in the NCAR CCSM4 climate model. J. Geophys. Res. Oceans, 118, 4755-4770, doi:10.1002/jgrc.20335.
Capotondi, A., and Coauthors, 2015: Understanding ENSO Diversity. Bull. Amer. Meteor. Soc., 96, 921-938, doi:10.1175/BAMS-D-13-00117.1.
Capotondi, A., and P. D. Sardeshmukh, 2015: Optimal precursors of different types of ENSO events. Geophys. Res. Lett., 42, doi:10.1002/2015GL066171.
Carton, J. A., and B. S. Giese, 2008: A reanalysis of ocean climate using simple ocean data assimilation (SODA). Mon. Wea. Rev., 136, 2999-3017, doi:10.1175/2007MWR1978.1.
Chiang, J. C. H., and D. J. Vimont, 2004: Analogous Pacific and Atlantic meridional modes of tropical atmosphere-ocean variability. J. Climate, 17, 4143-4158, doi: 10.1175/JCLI4953.1.
Deser, C., I. R. Simpson, K. A. McKinnon, and A. S. Phillips, 2017: The Northern Hemisphere extra-tropical atmospheric circulation response to ENSO: How well do we know it and how do we evaluate models accordingly? J. Climate, submitted.
Di Lorenzo, E., and Coauthors, 2008: North Pacific Gyre Oscillation links ocean climate and ecosystem change. Geophys. Res. Lett., 35, doi:10.1029/2007gl032838.
Di Lorenzo, E., and Coauthors, 2013: Synthesis of Pacific Ocean climate and ecosystem dynamics. Oceanogr., 26, 68-81, doi: 10.5670/oceanog.2013.76.
Fischer, J. L., W. T. Peterson, and R. R. Rykaczewski, 2015: The impact of El Niño events on the pelagic food chain in the northern California Current. Glob. Change Bio., 21, 4401-4414, doi:10.1111/gbc.13054.
Hermann, A. J., E. N. Curchitser, D. B. Haidvogel, and E. L. Dobbins, 2009. A comparison of remote vs. local influence of El Nino on the coastal circulation of the northeast Pacific. Deep Sea Res. Part II: Top. Stud. Oceanogr., 56, 2427-2443, doi: 10.1016/j.dsr2.2009.02.005.
Horel, J. D., and J. M. Wallace, 1981: Planetary-scale atmospheric phenomena associated with the Southern Oscillation. Mon. Wea. Rev., 109, 813-829, doi: 10.1175/1520-0493(1981)109<0813:PSAPAW>2.0.CO;2.
Huyer, A., R. L. Smith, and J. Fleischbein, 2002: The coastal ocean off Oregon and northern California during the 1997–8 El Nino. Prog. Oceanogr., 54, 311-341, doi: 10.1016/S0079-6611(02)00056-3.
Jacox, M. G., E. L. Hazen, K. D. Zaba, D. L. Rudnick, C. A. Edwards, A. M. Moore, and S. J. Bograd, 2016: Impacts of the 2015-2016 El Niño on the California Current System: Early assessment and comparison to past events. Geophys. Res. Lett., 43, 7072-7080, doi:10.1002/2016GL069716.
Jin, F.-F., 1997: An equatorial ocean recharge paradigm for ENSO. Part I: Conceptual model. J. Atmos. Sci., 54, 811-829, doi: 10.1175/1520-0469(1997)054<0811:AEORPF>2.0.CO;2.
Larkin, N. K., and D. E., Harrison, 2005: On the definition of El Niño and associated seasonal average U.S. weather anomalies. Geophys. Res. Lett. 32, doi:10.1029/2005GL022738.
Lynn, R. J., and S. J. Bograd, 2002: Dynamic evolution of the 1997–1999 El Niño–La Niña cycle in the southern California Current system. Prog. Oceanogr., 54, 59-75, doi: 10.1016/S0079-6611(02)00043-5..
Marchesiello, P., J. C. McWilliams, and A. Shchepetkin, 2003. Equilibrium structure and dynamics of the California Current System. J. Phys. Oceanogr., 33, 753-783, doi: 10.1175/1520-0485(2003)33<753:ESADOT>2.0.CO;2.
McPhaden, M. J., 1999: Climate oscillations – Genesis and evolution of the 1997-98 El Niño. Science, 283, 950-954.
Miller, A. J., H. Song, and A. C. Subramanian, 2015: The physical oceanographic environment during the CCE-LTER Years: Changes in climate and concepts. Deep Sea Res. Part II: Top. Stud. Oceanogr., 112, 6-17, doi: 10.1016/j.dsr2.2014.01.003.
Penland, C., and P. D. Sardeshmukh, 1995: The optimal growth of tropical sea surface temperature anomalies. J. Climate, 8, 1999-2024, doi: 10.1175/1520-0442(1995)008<1999:TOGOTS>2.0.CO;2.
Rasmusson, E. M., and T. H. Carpenter, 1982: Variations in tropical sea-surface temperature and surface wind fields associated with the Southern-Oscillation El Niño. Mon. Wea. Rev., 110, 354-384, doi: 10.1175/1520-0493(1982)110<0354:VITSST>2.0.CO;2.
Roundy, P. E., and G. N. Kiladis, 2006: Observed relationships between intraseasonal oceanic Kelvin waves and atmospheric forcing. J. Climate, 19, 5253-5272.
Simpson, J. J., 1984. El Nino‐induced onshore transport in the California Current during 1982‐1983. Geophys. Res. Lett., 11, 233-236, doi: 10.1029/GL011i003p00233.
Vimont, D. J., M. A. Alexander, and M. Newman, 2014: Optimal growth of central and east Pacific ENSO events. Geophys. Res. Lett., 41, doi:10.1002/2014GL059997.
Wyrtki, K., 1975: El Niño – The dynamic response of the equatorial Pacific Ocean to atmospheric forcing. J. Phys. Oceanogr., 5, 572-584, doi: 10.1175/1520-0485(1975)005<0572:ENTDRO>2.0.CO;2.
Xue, Y., and A. Kumar, 2016: Evolution of the 2015-16 El Niño and historical perspective since 1979. Sci. China, Earth Sci., doi:10.1007/s11430-016-0106-9.
Yu., J.-Y., and S. T. Kim, 2011: Relationships between extratropical sea level pressure variations and the central-Pacific and eastern-Pacific types of ENSO. J. Climate, 24, 708-720, doi: 10.1175/2010JCLI3688.1.
Zhang, H., A. Clement, and P. Di Nezio, 2014: The South Pacific Meridional Mode: A mechanism for ENSO-like variability. J. Climate, 27, 769-783, doi: 10.1175/JCLI-D-13-00082.1.